introduction
The central law of genetics holds that nucleic acid carry human genetic information and play a vital role in life processes such as growth, development and reproduction, and that nucleic acids can be used to modify genetic information to treat various diseases.
Nucleic acid drugs (NAD) are a class of gene therapy drugs based on DNA, RNA, or synthetic oligonucleotide analogues.
They have considerable potential for clinical applications, such as the treatment of bacterial infections, tumors and neuromuscular diseases, where long-term efficacy can be achieved through gene suppression, substitution and editing.
As an emerging therapeutic approach, NAD can precisely target specific genes or gene products to achieve precise treatment.
In addition, compared with traditional small molecule drugs and antibody drugs, the discovery and development cycle of NAD is shorter and the cost is relatively low, which helps accelerate the development of new drugs.
NAD not only has potential in the treatment of genetic diseases and cancer, but also can be applied to a variety of other diseases, such as cardiovascular diseases, metabolic diseases, neurodegenerative diseases, etc.
Therefore, the future prospects for NAD are bright and according to market analysis, the market size of NAD is expected to grow significantly in the next few years.
Historical development of NAD
The development of NAD is inseparable from the major discoveries of basic molecular biology and the continuous observation of life activities.
In 1869, Friedrich Miescher discovered a new molecule called “nuclide” from white blood cells, which marked the beginning of the discovery of DNA.
In 1956, Rich and Davies discovered that, based on the principle of complementary base pairing, RNA can form a double-stranded structure similar to DNA, a result that laid the foundation for the development of RNA double-stranded drugs, including microRNAs (miRNAs) and small interference RNAs (Sinas).
The double helix structure of DNA and the revelation of the central law of genetics have clarified that nucleic acids carry genetic information.
In 1960, Rich reported on DNA/RNA hybridization;
In 1978, building on this discovery, Zamecnik and Stephenson used a specific oligonucleotide chain targeting the 35S RNA of Rous sarcoma virus to inhibit viral replication, marking a prototype for the use of antisense oligonucleotide (ASO) drugs in disease treatment.
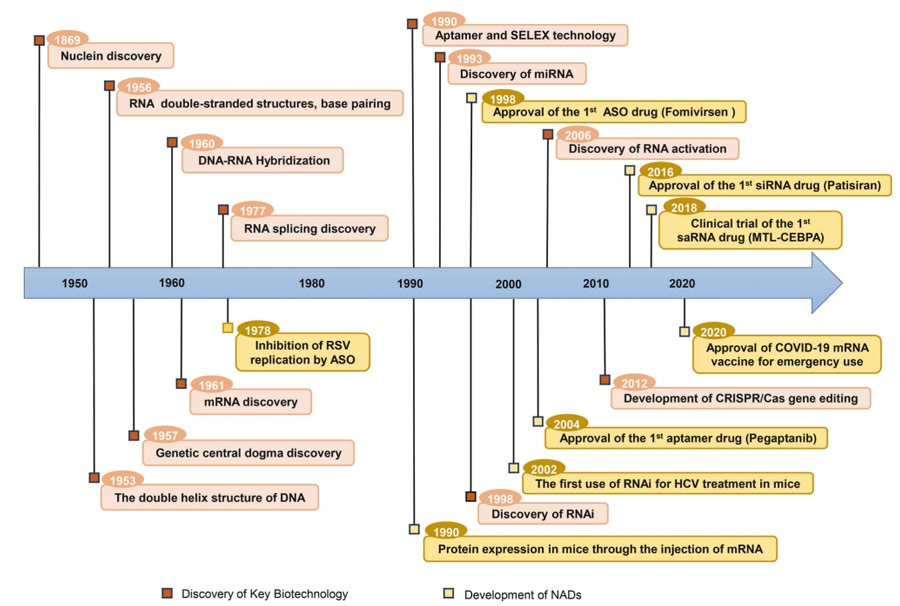
With the deepening of transcription and translation studies, the RNA splicing process has been discovered.
RNA splicing is a key step in gene expression, and ASO targeting splicing sites can restore the correct splicing of defective genes, rather than just down-regulating gene expression.
The findings suggest a new treatment strategy for diseases associated with missplicing.
In 1998, Andrew Fire and Craig Mello discovered that double-stranded RNA (dsRNA) has a powerful gene silencing effect in C. elegans, a finding they called RNA Interference (RNAi).
RNAi was rapidly applied to inhibit replication of hepatitis C virus in mice, marking the earliest evidence of SirNA-mediated gene silencing in vivo.
In 2006, they won the Nobel Prize in Physiology and Medicine for RNAi technology.
With the development of advanced chemical modifications and targeted delivery systems, in 2018 the U.S. Food and Drug Administration (FDA) approved patisiran, the first siRNA drug, for the treatment of polyneuropathy with inherited thyroxin-mediated amyloidosis.
In 1984, Krieg and Melton successfully achieved mRNA expression in cell-free systems using RNA polymerase extracted from viruses for in vitro transcription from DNA templates.
In 2005, the discovery of pseudouridine (Ψ) modification resolved the question of immunogenicity in the synthesis of mRNA in vitro, and the first human trial of an mRNA vaccine against melanoma was initiated in 2008.
As a result, mRNA synthesized in vitro has been increasingly used for protein replacement in preventive and therapeutic vaccines since the 1990s.
In 2020, the FDA authorized emergency use of mRNA vaccines during the COVID-19 pandemic.
In 2023, Katalin Kariko and Drew Weissman won the Nobel Prize in Physiology and Medicine for pioneering nucleoside base modification techniques that reduce mRNA immunogenics, which opened up new clinical applications for NAD in the treatment of human diseases.
At the same time, the discovery of other types of nucleic acids and biological phenomena further expanded the scope of NAD.
The emergence of gene editing technology has laid the foundation for the development of new therapies for diseases with genetic mutations.
Recently, the first gene therapy based on the clustered regularly spaced short palindromic repeat (CRISPR) /Cas9 technology has been approved for marketing, which has revolutionized the development of NAD.
Classification and therapeutic mechanism of NADs
According to its mechanism of action, NAD can be broadly divided into three categories:
The first category includes Nads that target nucleic acids to regulate protein expression by promoting or inhibiting translation.
- This category mainly includes ASO, siRNA, miRNA, saRNAs, and CRISPR/Cas systems;
- The second category includes NAds that target proteins, such as aptamers;
- The third category includes Nads that express proteins, such as mRNA transcribed in vitro.
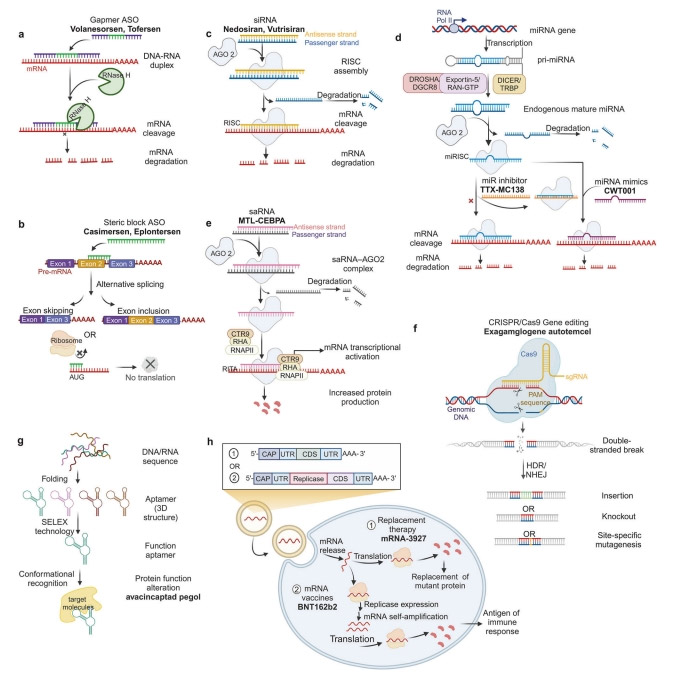
NAD targeting nucleic acids
ASO
ASO is a synthetic single-stranded oligonucleotide chain that regulates and targets RNA function by specific binding according to Watson-Crick base pairing principle.
The mechanism of action of ASO in clinical use mainly includes ribonuclease H (RNase H) mediated degradation and spatial blocking mechanisms.
Representative drugs based on this mechanism include Fomiversen (Vitravene®), Mipomersen (Kynamro®), Delisted), Inotersen (Tegesdi®), Volanesorsen (Waylivra®) and Tofersen (Qalsody®).
siRNA
siRNA is a double-stranded RNA molecule, usually 19-23 base pairs long, that induces gene silencing by blocking mRNA translation.
Unlike ASO, SirNA-mediated gene silencing is via an RNA-induced silencing complex (RISC) rather than RNase H.
Once the mature siRNA enters the cell, it forms a complex with the Argonaute-2 (AGO2) protein, which guides the RISC to cut the target sequence, thereby achieving therapeutic effects by down-regulating the translation of a specific protein.
To date, several siRNA drugs have received international approval. The first siRNA drug, patisiran (Onpattro®), has been approved to treat inherited transthyroxine mediated amyloidosis by degrading mRNA encoding transthyroxine protein (TTR).
miRNA
Endogenous non-coding Rnas found in eukaryotes can act as gene regulators.
The regulatory mechanisms of mirnas have extensively studied, and primary mirnas (PRI-mirnas) transcribed from non-coding regions of miRNA genes processed into mature mirnas with the help of complexes such as Drosha/DGCR, Exportin-5/RAN-GTP, and Dicer/TRBP.
Mirnas bind to Argonaute proteins to form mirNA-induced silencing complexes (miRISC), which can silence target transcripts by complementing base pairs.
Unlike SIRNA-mediated gene silencing, mirnas can simultaneously recognize and regulate the expression of multiple target mrnas because they are less complementary.
The development of miRNA drugs mainly includes two categories: miRNA mimics and miRNA inhibitors.
The miRNA drug MRG-201 (Remlarsen) developed by Viridian Therapeutics mimics microRNA-29b to reverse fibrosis, thereby inhibiting fiber proliferation in skin wounds.
In addition to MRG-201, Viridian Therapeutics has several other mirNa-based drugs, including MRG-106 (Cobomarsen), which targets miR-155 for tumor suppression;
MRG-110, which targets miR-92 to promote angiogenesis.
To date, no miRNA drugs have approved for marketing.
saRNA
In addition to classical gene silencing mechanisms, the discovery of RNA activation provides new perspectives on gene regulation.
RNA activation a phenomenon of gene regulation mediated by small Dsrnas called SARnas,
which target gene promoter sequences to enhance transcription of target genes.
After entering the cell through endocytosis, the saRNA binds to AGO2,
and the AGO2 and antisense chains transported to the nucleus, where they bind to the promoter region.
Subsequently, AGO2 recruits the RNA polymerase-associated protein CTR9 homologues and RNA helicase II (RHA) to form RNA-induced transcriptional activation (RITA) complexes.
This complex interacts with RNA polymerase II (RNAP II) and stimulates the initiation and elongation of transcription.
The saRNA drug McL-cepa developed by MiNA Therapeutics targets CCAAT/ enhancer binding protein alpha (CEBPA).
Phase I trial results (NCT05097911) suggest that McL-cebapa has a good safety profile in the treatment of hepatocellular carcinoma and may enhance the therapeutic efficacy of tyrosine kinase inhibitors by modulating immunosuppression.
In addition to McL-cempa, MiNA Therapeutics has several saRNA candidates in preclinical development.
The CRISPR/Cas9 system
Gene editing is a genetic engineering technique that precisely modifies target genes through insertions, deletions, and site-specific mutations.
Crispr-mediated gene editing systems primarily involve Cas9 and single-directed Rnas (Sgrnas) that introduce DNA double-strand breaks (DSBs) at specific locations in the genome.
These DSBS usually repaired by homologous directed repair (HDR) or non-homologous end junction (NHEJ), enabling mutation or foreign gene insertion.
Exa-cel (Casgevy®), approved in November 2023, the first CRISPR/Cass9 system-based gene editing therapy for the treatment of sickle cell disease (SCD) and transfusion dependent beta-thalassemia (TDT).
NAD targeting proteins
Aptamers single-stranded oligonucleotide molecules that, unlike other nucleic acid-based drugs,
rely on their unique three-dimensional conformation specificity to recognize and bind to target molecules such as peptides, proteins, viruses, bacteria, and cells.
This is similar to conformational recognition that mediates antibody-antigen interactions and complex formation.
However, aptamers have several advantages over antibodies, including high thermal and physiological stability, low immunogenicity, and broader target specificity.
Aptamers have shown promising applications in the treatment of cancer, ophthalmology and cardiovascular disease (CVD).
In 2004, the FDA approved pegaptanib (Macugen®), the first aptamer drug, for the treatment of neovascular age-related macular degeneration (AMD).
However, pegaptanib withdrawn from the market due to its poor efficacy and competition with anti-VEGF antibody drugs such as Lucentis.
Nevertheless, research on aptamers continues.
In August 2023, avacincaptad pegol approved for the treatment of map-like atrophy (GA) secondary to dry AMD.
Expression of protein NAD
In contrast to NAD, which acts therapeutically by directly binding mRNA or protein, mRNA drugs have two therapeutic strategies.
One strategy is protein replacement therapy, which involves introducing exogenous mRNAs into cells to express functional proteins or to supplement defective proteins.
For example, mRNA-3927 encodes the alpha and beta subunits of propionyl-CoA carboxylase, which designed to treat propionicemia.
A Phase I/II trial (NCT05130437) for this indication has initiated in pediatric patients.
In addition, mRNA-3704 and mRNA-3705 encode MUT, which designed to treat methylmalonic acidemia and are currently being studied.
The second approach involves mRNA vaccines, which activate the body’s immune response by directly translating mRNA containing antigen proteins to fight infectious diseases and tumors.
During the COVID-19 pandemic, the FDA authorized emergency use of BNT162b2, developed by Pfizer-Biontech, and mRNA-1273, developed by Moderna.
Challenges of NAD development
Unlike traditional small molecule and antibody drugs, which act pharmacologically on proteins,
most Nads directly regulate gene expression, providing a wider range of targets.
This is especially valuable for addressing genes with defective proteins that are difficult to target with traditional drugs,
showing great potential in treating rare diseases, chronic diseases, infectious diseases and other metabolic diseases.
However, despite these advantages, the development of NAD still faces a series of challenges.
Pharmacokinetics and stability
Naked nucleic acids less stable in the body and can degraded in the blood.
They undergo enzymatic (nuclease and RNase) or chemical (oxidative and hydrolytic) degradation in the blood and interstitial fluid, or filtered and cleared by the kidneys.
Although researchers have enhanced the stability of NAD through several chemical modifications,
almost all of these methods affect efficacy and safety.
immunogenicity
Foreign nucleic acids can recognized as foreign signals by pattern recognition receptors in the immune system,
triggering an immune response that impairs the structural integrity and stability of nucleic acids.
Careful design and modification of NAD can mitigate immunogenicity, but this requires extensive clinical testing.
Off-target effect
Therapeutic nucleic acids often lack sufficient targeting ability in vivo.
This inadequate targeting results in low concentrations of NAD at the disease site,
as well as unexpected gene silencing or activation,
which can lead to safety issues due to the need for higher doses.
Designing specific nucleic acids and conducting thorough off-target screening are critical for effective clinical use of NAD.
Uptake efficiency and endosomal escape
Due to the size, charge, and hydrophilicity of NAD, it is difficult to effectively absorb it into cells.
Negatively charged nucleic acids do not easily cross the negatively charged lipid bilayer of the cell membrane.
In addition, endosome escape an important obstacle because nucleic acids often get trapped in endosomes and degrade.
Once inside the cell, NAD usually captured by endosomes.
Only those NAds that have managed to escape the endosome can exert their therapeutic effects.
Insufficient endosomal escape can lead to reduced efficacy and increased off-target toxicity.
manufacturing
In addition to the previous issues, NAD faces challenges in manufacturing and scaling up.
Mass production of NAD with consistent quality requires advanced manufacturing techniques and strict quality control measures.
This results in relatively high costs, impacting patient access and affordability.
Sum up
Nucleic acid drug based therapies are emerging as potential intervention strategies for various diseases.
In general, NAD therapies fall into different categories based on how they work and the molecules they use.
A number of NAD therapies have developed for a variety of diseases and have shown very promising results in many preclinical and clinical studies,
with mRNA vaccines in particular becoming one of the hottest research areas at the moment.
At present, there are still many challenges in NAD-based therapies,
including many aspects of the delivery system still need to be further research and development,
but there is no denying that the next decade belongs to the era of NAD therapy will come.
References:
- 1. RNA Therapeutics – Research and Clinical Advancements. Front Mol Biosci. 2021; 8: 710738.
- 2. mRNA vaccines for infectious diseases: principles, delivery and clinical translation. Nat RevDrugDiscov. 2021 Aug 25 : 1–22.
- 3. Nucleic acid drugs: recent progress and future perspectives. Signal Transduct Target Ther. 2024 Nov 29;9:316